Gyrification

Gyrification is the process of forming the characteristic folds of the cerebral cortex.[1] The peak of such a fold is called a gyrus (plural: gyri), and its trough is called a sulcus (plural: sulci). The neurons of the cerebral cortex reside in a thin layer of 'gray matter', only 2–4 mm thick, at the surface of the brain.[2] Much of the interior volume is occupied by 'white matter', which consists of long axonal projections to and from the cortical neurons residing near the surface. Gyrification allows a larger cortical surface area and hence greater cognitive functionality to fit inside a smaller cranium. In most mammals, gyrification begins during fetal development. Primates, cetaceans, and ungulates have extensive cortical gyri, with a few species exceptions, while rodents generally have none. Gyrification in some animals, for example the ferret, continues well into postnatal life.[3]
Gyrification during human brain development
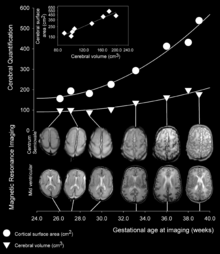
As fetal development proceeds, gyri and sulci begin to take shape with the emergence of deepening indentations on the surface of the cortex. Not all gyri begin to develop at the same time. Instead, the primary cortical gyri form first (beginning as early as gestational week 10 in humans), followed by secondary and tertiary gyri later in development.[4] One of the first and most prominent sulci is the lateral sulcus (also known as the lateral fissure or Sylvian fissure), followed by others such as the central sulcus, which separates the motor cortex (precentral gyrus) from somatosensory cortex (postcentral gyrus).[5] Most cortical gyri and sulci begin to take shape between weeks 24 and 38 of gestation, and continue to enlarge and mature after birth.
Evolutionary advantages
One advantage of gyrification is thought to be increased speed of brain cell communication, since cortical folds allow for cells to be closer to one other, requiring less time and energy to transmit neuronal electrical impulses, termed action potentials.[6] There is evidence to suggest a positive relationship between gyrification and cognitive information processing speed, as well as better verbal working memory.[7] Additionally, because a large cranium requires a larger pelvis during childbirth, with implied difficulty in bipedalism, a smaller cranium is more easily delivered.[8][9]
Theories on causality in gyrification
Mechanical buckling
The mechanisms of cortical gyrification are not well understood, and several hypotheses are debated in the scientific literature. A popular hypothesis dating back to the time of Retzius in the late 19th century asserts that mechanical buckling forces due to the expanding brain tissue cause the cortical surface to fold.[10] Many theories since have been loosely tied to this hypothesis.
An external growth constraint of the cranium is not thought to cause gyrification. This is principally because the primordium of the cranium during the period of fetal brain development is not yet ossified (hardened into bone through calcification). The tissue covering the embryonic cerebral cortex is several thin layers of ectoderm (future skin) and mesenchyme (future muscle and connective tissue, including the future cranium). These thin layers of grow easily along with cortical expansion but eventually the cranial mesenchyme differentiates into cartilage; ossification of the cranial plates does not occur until later in development. The human cranium continues to grow substantially along with the brain after birth until the cranial plates finally fuse after several years. Experimental studies in animals have furthermore shown that cortical folding can occur without external constraints.[11] Cranial growth is thus thought to be driven by brain growth; mechanical and genetic factors intrinsic to the brain are now thought to be the primary drivers of gyrification.[6] The only observed role that the cranium may play in gyrification is in flattening of gyri as the brain matures after the cranial plates fuse.[11]
Axonal tension
An alternative theory suggests that axonal tension forces between highly interconnected cortical areas pull local cortical areas towards each other, inducing folds.[12] There is some evidence to support this hypothesis. For example, axons in areas of the brain with high gyrification levels are straight, short in length, and found to connect gyral walls. This evidence, however, cannot be determined to be a cause or result of cortical folding. While short connections may be a result of axons pulling on the walls of forming gyri, the axons may adaptively remain short due to mechanical growth patterns.[6] Additionally, one study showed that gyrification can be experimentally induced in the embryonic mouse, but at early stages in the absence of axonal connections.[13]
Differential tangential expansion
More recently, the theory of differential tangential expansion has been proposed, stating that folding patterns of the brain are a result of different tangential expansion rates between different cortical areas.[14] This is proposed to be due to areal differences in early progenitor division rates.
Mechanical factors
Cortical thickness
Early conditions of the brain have a strong influence on its final level of gyrification. In particular, there is an inverse relationship between cortical thickness and gyrification. Areas of the brain with low values of thickness are found to have higher levels of gyrification. The reverse is also true, that areas of the brain with high values of thickness are found to have lower levels of gyrification.[6]
Growth speed
There is some dispute over the growth rates through which cortical and subcortical layers of the brain develop. Purely isotropic growth suggests that the grey (outer shell) and white matter (inner core) layers each grow at separate rates, that are uniform in all dimensions. Tangential growth suggests that the grey matter grows at a faster rate than the inner white matter, and that the growth rate of the grey matter determines the growth rate of the white matter. Though both methods are differential, with the cortex growing more rapidly than the subcortex, tangential growth has been suggested as a more plausible model.[6]
Creases on the brain's surface are formed as a result of instability, and tangential growth models reach levels of instability that cause creasing more frequently than isotropic models. This level is called a critical point, at which, the models prefer to release potential energy by destabilizing and forming creases to become more stable.[6]
Genetic factors
The pattern of cortical gyri and sulci is not random; most of the major convolutions are conserved between individuals and many are also found across species. This reproducibility may suggest that genetic mechanisms can specify the location of major gyri. Studies of monozygotic and dizygotic twins of the late 1990s support this idea,[15] particularly with regards to primary gyri and sulci, whereas there is more variability among secondary and tertiary gyri.[16] Therefore, one may hypothesize that secondary and tertiary folds could be more sensitive to genetic and environmental factors.[17] Genes that influence cortical progenitor dynamics, neurogenesis and neuronal migration, as well as genes that influence the development of cortical circuits and axonal projections may all contribute to gyrification. Indeed, several genes have been shown to induce artificial gyri and sulci in mouse models. The Fibroblast Growth Factor (FGF)- and Sonic Hedgehog (SHH)-signaling pathways have recently been reported to be able to induce healthy cortical folds, with a full complement of cortical layers, in mice that live to adulthood.[13][18] These FGF and Shh factors regulate cortical stem cell proliferation and neurogenesis dynamics. In addition, local expression levels of another gene, Trnp1, can determine the future position of a developing fold/gyrus.[19][20][21] Trnp1 is a DNA-binding factor that has been shown to regulate other genes that regulate the proliferation of cortical progenitor cells including radial glial cells.[6][19] Roles for beta-catenin (part of the Wnt pathway) and appropriate levels of cell death of cortical progenitors have also been found.[22][23]
Cell biological determinants
Cortical stem cells, known as radial glial cells (RGC)s, reside in the ventricular zone and generate the excitatory glutamatergic neurons of the cerebral cortex.[24][25] These cells rapidly proliferate through self-renewal at early developmental stages, expanding the progenitor pool and increasing cortical surface area. At this stage, the pattern of cortical areas is genetically programmed by a system of signaling centers through the process of cortical patterning, and the primordial map of cortical functional areas at this stage is called a 'protomap'.[26] Cortical neurogenesis begins to deplete the pool of progenitor cells, subject to the influences of many genetic cues such as fibroblast growth factors (FGF)s and Notch.[27] RGCs generate intermediate neuronal precursors that divide further in the subventricular zone (SVZ), amplifying the number of cortical neurons being produced.[28] The long fibers of RGCs project all the way through the developing cortex to the pial surface of the brain, and these fibers serve as physical guides for neuronal migration.[29] A second class of RGC, termed basal RGCs (bRGC)s, forms a third progenitor pool in the outer SVZ.[30] Basal RGCs are generally much more abundant in higher mammals. Both classic RGCs and the recently described bRGCs represent guiding cues that lead newborn neurons to their destination in the cortex. Increased numbers of bRGCs increase the density of guiding fibers in an otherwise fanning out array which would lose fiber density.[31] The scientific literature points to differences in the dynamics of proliferation and neuronal differentiation in each of these progenitor zones across mammalian species, and such differences may account for the large differences in cortical size and gyrification among mammals. One hypothesis suggests that certain progenitor cells generate abundant neurons destined for the outer cortical layers, causing greater surface area increase in the outer layers compared with the inner cortical layers.[31] It remains unclear how this may work without further mechanistic elements.[32][33]
Variation across species
A 'gyrification index' (GI) has been used as a measure of the magnitude of cortical convolutions on the surface of the mammalian brain.[34][35] Reptile and bird brains do not show gyrification. Mammals with a high GI are generally larger than those with a low GI; for example the pilot whale and bottlenose dolphin show the highest GI values. The human brain, while larger than that of a horse, shows a similar GI. Rodents generally show the lowest GIs. Nonetheless, some rodents show gyrencephaly and a few primate species are quite lissencephalic.[36]
Neurological disorders of gyrification
Lissencephaly
A cerebral cortex lacking surface convolutions is said to be lissencephalic, meaning 'smooth-brained'.[37] During embryonic development, all mammalian brains begin as lissencephalic structures derived from the neural tube. Some, like mouse brains, remain lissencephalic throughout adulthood. It has been shown that lissencephalic species possess many of the molecular cues needed to achieve gyrencephaly, but a large variety of genes are involved in the regulation of the neural progenitor proliferation and neurogenic processes that underlie gyrification. It is hypothesized that spatiotemporal differences in these molecular pathways, including FGF, Shh, and Trnp1 and likely many others, determine the timing and extent of gyrification in various species.[19][38]
Lissencephaly is a human disease state. For humans with lissencephaly, a large portion of neurons fail to reach the outer cortex during neuronal migration, and remain under the cortical plate.[39] This displacement results in not only defects in cortical connections, but also a thickened cortex, consistent with the idea that a brain with a thicker cortex will have a lesser degree of gyrification.[40]
Polymicrogyria
Polymicrogyria is a condition in which the brain has an overly convoluted cortex. Though at the surface, the brain appears smooth with a few sulci, looking at the interior of the brain reveals a convoluted structure with a large number of secondary and tertiary folds.[40] Brain imaging with MRI reveals a brain with polymicrogyria to have a thin cortex, consistent with the idea that a brain with a thin cortex will have a high level of gyrification.[39][40]
Autism
Patients with autism have overall higher levels of cortical gyrification,[41] but only in the temporal, parietal, and occipital lobes, as well as part of the cingulate cortex.[42] The higher levels of gyrification are found to relate to greater local connectivity in autistic brains, suggesting hyperconnectivity.
Trnp1, which was able to induce gyrification in animal models was also identified in patients with autism spectrum diseases, thus indicating a molecular role of Trnp1 in autism.[43]
The folds of autistic human brains are found to experience slight shifts in location, early brain development. Specifically, different patterns appear in the superior frontal sulcus, Sylvian fissure, inferior frontal gyrus, superior temporal gyrus, and olfactory sulci.[44] These areas relate to working memory, emotional processing, language, and eye gaze,[45] and their difference in location and level of gyrification when compared to a healthy human brain could explain some altered behaviors in autistic patients.
Schizophrenia
A more prevalent condition, schizophrenia, has been associated with structural abnormalities in the brain as well. Like autistic brains, schizophrenic brains show reduced cortical thickness and increased gyrification when compared to healthy brains.[39][46]
Zika virus malformations
Cortical malformations induced by the Zika virus are due to infection during pregnancy, and are generally classified as microcephaly, or 'small-brain'. Due to the large reduction in volume of the cerebral cortex in microcephaly, changes in gyrification are not unexpected. However, recent studies of the mechanism of Zika malformations indicate that the principal defect is due to infection of RGCs and subsequent cell death.[47][48] Death of cortical stem cells causes the loss of all expected daughter cells, and the scope of the malformation thus depends on the timing of infection as well as its severity during the schedule of neural stem cell proliferation and neurogenesis. Earlier infections would generally be expected to produce a more severe malformation.[49][50] The microcephaly and gyrification malformations are permanent and there are no known treatments.
Additional images
- Various brains. Clockwise from top left: Adult rhesus; Adult mouse; Midgestation human; Newborn human; Adult human.
- Normal human adult cerebrum (left), polymicrogyria (center) and lissencephaly (right).
References
- ↑ Rakic, P (October 2009). "Evolution of the neocortex: a perspective from developmental biology.". Nature reviews. Neuroscience. 10 (10): 724–35. doi:10.1038/nrn2719. PMC 2913577
. PMID 19763105.
- ↑ Principles of neural science (5. ed.). Appleton and Lange: McGraw Hill. 2006. ISBN 978-0071390118.
|first1=
missing|last1=
in Authors list (help) - ↑ Smart, IH; McSherry, GM (June 1986). "Gyrus formation in the cerebral cortex in the ferret. I. Description of the external changes.". Journal of Anatomy. 146: 141–52. PMC 1166530
. PMID 3693054.
- ↑ Rajagopalan, V; Scott, J; Habas, PA; Kim, K; Corbett-Detig, J; Rousseau, F; Barkovich, AJ; Glenn, OA; Studholme, C (23 February 2011). "Local tissue growth patterns underlying normal fetal human brain gyrification quantified in utero.". The Journal of neuroscience : the official journal of the Society for Neuroscience. 31 (8): 2878–87. doi:10.1523/jneurosci.5458-10.2011. PMID 21414909.
- ↑ Bayer, Shirley A; Altman, Joseph (2005). The Human Brain during the Second Trimester. Boca Raton, FL USA: CRC Press. ISBN 978-0-8493-1422-3.
- 1 2 3 4 5 6 7 Striedter, Georg F.; Srinivasan, Shyam; Monuki, Edwin S. (2015-01-01). "Cortical Folding: When, Where, How, and Why?". Annual Review of Neuroscience. 38 (1): 291–307. doi:10.1146/annurev-neuro-071714-034128. PMID 25897870.
- ↑ Gautam, Prapti; Anstey, Kaarin J.; Wen, Wei; Sachdev, Perminder S.; Cherbuin, Nicolas (2015-07-01). "Cortical gyrification and its relationships with cortical volume, cortical thickness, and cognitive performance in healthy mid-life adults". Behavioural Brain Research. 287: 331–339. doi:10.1016/j.bbr.2015.03.018.
- ↑ Jordaan, HV (March 1976). "Newborn: adult brain ratios in hominid evolution.". American Journal of Physical Anthropology. 44 (2): 271–8. doi:10.1002/ajpa.1330440209. PMID 816206.
- ↑ Weiner, S; Monge, J; Mann, A (September 2008). "Bipedalism and parturition: an evolutionary imperative for cesarean delivery?". Clinics in perinatology. 35 (3): 469–78, ix. doi:10.1016/j.clp.2008.06.003. PMID 18952015.
- ↑ Ronan, L; Voets, N; Rua, C; Alexander-Bloch, A; Hough, M; Mackay, C; Crow, TJ; James, A; Giedd, JN; Fletcher, PC (August 2014). "Differential tangential expansion as a mechanism for cortical gyrification.". Cerebral cortex (New York, N.Y. : 1991). 24 (8): 2219–28. doi:10.1093/cercor/bht082. PMID 23542881.
- 1 2 Tallinen, Tuomas; Chung, Jun Young; Biggins, John S.; Mahadevan, L. (2014-09-02). "Gyrification from constrained cortical expansion". Proceedings of the National Academy of Sciences. 111 (35): 12667–12672. doi:10.1073/pnas.1406015111. ISSN 0027-8424. PMC 4156754
. PMID 25136099.
- ↑ Van Essen, DC (23 January 1997). "A tension-based theory of morphogenesis and compact wiring in the central nervous system.". Nature. 385 (6614): 313–8. doi:10.1038/385313a0. PMID 9002514.
- 1 2 Rash, BG; Tomasi, S; Lim, HD; Suh, CY; Vaccarino, FM (26 June 2013). "Cortical gyrification induced by fibroblast growth factor 2 in the mouse brain.". The Journal of neuroscience : the official journal of the Society for Neuroscience. 33 (26): 10802–14. doi:10.1523/jneurosci.3621-12.2013. PMC 3693057
. PMID 23804101.
- ↑ Ronan, L; Voets, N; Rua, C; Alexander-Bloch, A; Hough, M; Mackay, C; Crow, TJ; James, A; Giedd, JN; Fletcher, PC (August 2014). "Differential tangential expansion as a mechanism for cortical gyrification.". Cerebral cortex (New York, N.Y. : 1991). 24 (8): 2219–28. doi:10.1093/cercor/bht082. PMID 23542881.
- ↑ Bartley, AJ; Jones, DW; Weinberger, DR (February 1997). "Genetic variability of human brain size and cortical gyral patterns.". Brain : a journal of neurology. 120 (2): 257–69. doi:10.1093/brain/120.2.257. PMID 9117373.
- ↑ White, Tonya; Su, Shu; Schmidt, Marcus; Kao, Chiu-Yen; Sapiro, Guillermo (2010-02-01). "The development of gyrification in childhood and adolescence". Brain and Cognition. Adolescent Brain Development: Current Themes and Future Directions. 72 (1): 36–45. doi:10.1016/j.bandc.2009.10.009. PMC 2815169
. PMID 19942335.
- ↑ Gómez-Robles, Aida; Hopkins, William D.; Sherwood, Chet C. (2013-06-22). "Increased morphological asymmetry, evolvability and plasticity in human brain evolution". Proc. R. Soc. B. 280 (1761): 20130575. doi:10.1098/rspb.2013.0575. ISSN 0962-8452. PMC 3652445
. PMID 23615289.
- ↑ Wang, L; Hou, S; Han, YG (23 May 2016). "Hedgehog signaling promotes basal progenitor expansion and the growth and folding of the neocortex.". Nature Neuroscience. 19: 888–96. doi:10.1038/nn.4307. PMID 27214567.
- 1 2 3 Stahl, Ronny; Walcher, Tessa; De Juan Romero, Camino; Pilz, Gregor Alexander; Cappello, Silvia; Irmler, Martin; Sanz-Aquela, José Miguel; Beckers, Johannes; Blum, Robert (2013-04-25). "Trnp1 regulates expansion and folding of the mammalian cerebral cortex by control of radial glial fate". Cell. 153 (3): 535–549. doi:10.1016/j.cell.2013.03.027. ISSN 1097-4172. PMID 23622239.
- ↑ de Juan Romero, Camino; Bruder, Carl; Tomasello, Ugo; Sanz-Anquela, José Miguel; Borrell, Víctor (2015-07-14). "Discrete domains of gene expression in germinal layers distinguish the development of gyrencephaly". The EMBO Journal. 34 (14): 1859–1874. doi:10.15252/embj.201591176. ISSN 1460-2075. PMC 4547892
. PMID 25916825.
- ↑ Fernández, Virginia; Llinares-Benadero, Cristina; Borrell, Víctor (2016-05-17). "Cerebral cortex expansion and folding: what have we learned?". The EMBO Journal. 35 (10): 1021–1044. doi:10.15252/embj.201593701. ISSN 1460-2075. PMC 4868950
. PMID 27056680.
- ↑ Chenn, A; Walsh, CA (19 July 2002). "Regulation of cerebral cortical size by control of cell cycle exit in neural precursors.". Science. 297 (5580): 365–9. doi:10.1126/science.1074192. PMID 12130776.
- ↑ Kuida, K; Haydar, TF; Kuan, CY; Gu, Y; Taya, C; Karasuyama, H; Su, MS; Rakic, P; Flavell, RA (7 August 1998). "Reduced apoptosis and cytochrome c-mediated caspase activation in mice lacking caspase 9.". Cell. 94 (3): 325–37. doi:10.1016/s0092-8674(00)81476-2. PMID 9708735.
- ↑ Noctor, SC; Flint, AC; Weissman, TA; Dammerman, RS; Kriegstein, AR (8 February 2001). "Neurons derived from radial glial cells establish radial units in neocortex.". Nature. 409 (6821): 714–20. doi:10.1038/35055553. PMID 11217860.
- ↑ Malatesta, P; Hartfuss, E; Götz, M (December 2000). "Isolation of radial glial cells by fluorescent-activated cell sorting reveals a neuronal lineage.". Development (Cambridge, England). 127 (24): 5253–63. PMID 11076748.
- ↑ Rakic, P (8 July 1988). "Specification of cerebral cortical areas.". Science (New York, N.Y.). 241 (4862): 170–6. PMID 3291116.
- ↑ Rash, BG; Lim, HD; Breunig, JJ; Vaccarino, FM (26 October 2011). "FGF signaling expands embryonic cortical surface area by regulating Notch-dependent neurogenesis.". The Journal of neuroscience : the official journal of the Society for Neuroscience. 31 (43): 15604–17. doi:10.1523/jneurosci.4439-11.2011. PMID 22031906.
- ↑ Noctor, SC; Martínez-Cerdeño, V; Ivic, L; Kriegstein, AR (February 2004). "Cortical neurons arise in symmetric and asymmetric division zones and migrate through specific phases.". Nature Neuroscience. 7 (2): 136–44. doi:10.1038/nn1172. PMID 14703572.
- ↑ Rakic, P (May 1972). "Mode of cell migration to the superficial layers of fetal monkey neocortex.". The Journal of Comparative Neurology. 145 (1): 61–83. doi:10.1002/cne.901450105. PMID 4624784.
- ↑ LaMonica, BE; Lui, JH; Wang, X; Kriegstein, AR (October 2012). "OSVZ progenitors in the human cortex: an updated perspective on neurodevelopmental disease.". Current Opinion in Neurobiology. 22 (5): 747–53. doi:10.1016/j.conb.2012.03.006. PMID 22487088.
- 1 2 Hansen, DV; Lui, JH; Parker, PR; Kriegstein, AR (25 March 2010). "Neurogenic radial glia in the outer subventricular zone of human neocortex.". Nature. 464 (7288): 554–561. doi:10.1038/nature08845. PMID 20154730.
- ↑ Hevner, RF; Haydar, TF (February 2012). "The (not necessarily) convoluted role of basal radial glia in cortical neurogenesis.". Cerebral cortex (New York, N.Y. : 1991). 22 (2): 465–8. doi:10.1093/cercor/bhr336. PMID 22116731.
- ↑ García-Moreno, F; Vasistha, NA; Trevia, N; Bourne, JA; Molnár, Z (February 2012). "Compartmentalization of cerebral cortical germinal zones in a lissencephalic primate and gyrencephalic rodent.". Cerebral cortex (New York, N.Y. : 1991). 22 (2): 482–92. doi:10.1093/cercor/bhr312. PMID 22114081.
- ↑ Striedter, GF; Srinivasan, S; Monuki, ES (8 July 2015). "Cortical folding: when, where, how, and why?". Annual Review of Neuroscience. 38: 291–307. doi:10.1146/annurev-neuro-071714-034128. PMID 25897870.
- ↑ Zilles, K; Armstrong, E; Moser, KH; Schleicher, A; Stephan, H (1989). "Gyrification in the cerebral cortex of primates.". Brain, behavior and evolution. 34 (3): 143–50. PMID 2512000.
- ↑ Hevner, RF; Haydar, TF (February 2012). "The (not necessarily) convoluted role of basal radial glia in cortical neurogenesis.". Cerebral cortex (New York, N.Y. : 1991). 22 (2): 465–8. doi:10.1093/cercor/bhr336. PMID 22116731.
- ↑ Armstrong, E; Schleicher, A; Omran, H; Curtis, M; Zilles, K (1991). "The ontogeny of human gyrification.". Cerebral cortex (New York, N.Y. : 1991). 5 (1): 56–63. PMID 7719130.
- ↑ Wang, Lei; Hou, Shirui; Han, Young-Goo (2016-05-23). "Hedgehog signaling promotes basal progenitor expansion and the growth and folding of the neocortex". Nature Neuroscience. 19: 888–96. doi:10.1038/nn.4307. ISSN 1546-1726. PMID 27214567.
- 1 2 3 Budday, Silvia; Raybaud, Charles; Kuhl, Ellen (2014-07-10). "A mechanical model predicts morphological abnormalities in the developing human brain". Scientific Reports. 4. doi:10.1038/srep05644. PMC 4090617
. PMID 25008163.
- 1 2 3 Ross, M. Elizabeth; Walsh, Christopher A. (2001-01-01). "Human Brain Malformations and Their Lessons for Neuronal Migration". Annual Review of Neuroscience. 24 (1): 1041–1070. doi:10.1146/annurev.neuro.24.1.1041. PMID 11520927.
- ↑ Wallace, Gregory L.; Robustelli, Briana; Dankner, Nathan; Kenworthy, Lauren; Giedd, Jay N.; Martin, Alex (2013-06-01). "Increased gyrification, but comparable surface area in adolescents with autism spectrum disorders". Brain. 136 (6): 1956–1967. doi:10.1093/brain/awt106. ISSN 0006-8950. PMC 3673467
. PMID 23715094.
- ↑ Yang, Daniel Y.-J.; Beam, Danielle; Pelphrey, Kevin A.; Abdullahi, Sebiha; Jou, Roger J. (2016-01-25). "Cortical morphological markers in children with autism: a structural magnetic resonance imaging study of thickness, area, volume, and gyrification". Molecular Autism. 7 (1). doi:10.1186/s13229-016-0076-x. PMC 4727390
. PMID 26816612.
- ↑ Stahl, Ronny (2012). Identification and functional analysis of Trnp1: a novel DNA associated protein with a key role in neurogenesis. https://edoc.ub.uni-muenchen.de/15432/. pp. 86–88.
- ↑ Chen, Jason A.; Peñagarikano, Olga; Belgard, T. Grant; Swarup, Vivek; Geschwind, Daniel H. (2015-01-01). "The Emerging Picture of Autism Spectrum Disorder: Genetics and Pathology". Annual Review of Pathology: Mechanisms of Disease. 10 (1): 111–144. doi:10.1146/annurev-pathol-012414-040405. PMID 25621659.
- ↑ Levitt, Jennifer G.; Blanton, Rebecca E.; Smalley, Susan; Thompson, P. M.; Guthrie, Donald; McCracken, James T.; Sadoun, Tania; Heinichen, Laura; Toga, Arthur W. (2003-07-01). "Cortical Sulcal Maps in Autism". Cerebral Cortex. 13 (7): 728–735. doi:10.1093/cercor/13.7.728. ISSN 1047-3211. PMID 12816888.
- ↑ Palaniyappan, Lena; Mallikarjun, Pavan; Joseph, Verghese; White, Thomas P.; Liddle, Peter F. "Folding of the Prefrontal Cortex in Schizophrenia: Regional Differences in Gyrification". Biological Psychiatry. 69 (10): 974–979. doi:10.1016/j.biopsych.2010.12.012.
- ↑ Nowakowski, TJ; Pollen, AA; Di Lullo, E; Sandoval-Espinosa, C; Bershteyn, M; Kriegstein, AR (5 May 2016). "Expression Analysis Highlights AXL as a Candidate Zika Virus Entry Receptor in Neural Stem Cells.". Cell stem cell. 18 (5): 591–6. doi:10.1016/j.stem.2016.03.012. PMID 27038591.
- ↑ Li, C; Xu, D; Ye, Q; Hong, S; Jiang, Y; Liu, X; Zhang, N; Shi, L; Qin, CF; Xu, Z (11 May 2016). "Zika Virus Disrupts Neural Progenitor Development and Leads to Microcephaly in Mice.". Cell stem cell. 19: 120–6. doi:10.1016/j.stem.2016.04.017. PMID 27179424.
- ↑ Wu, Kong-Yan; Zuo, Guo-Long; Li, Xiao-Feng; Ye, Qing; Deng, Yong-Qiang; Huang, Xing-Yao; Cao, Wu-Chun; Qin, Cheng-Feng; Luo, Zhen-Ge (2016-05-13). "Vertical transmission of Zika virus targeting the radial glial cells affects cortex development of offspring mice". Cell Research. 26: 645–654. doi:10.1038/cr.2016.58. ISSN 1748-7838.
- ↑ Tang, Hengli; Hammack, Christy; Ogden, Sarah C.; Wen, Zhexing; Qian, Xuyu; Li, Yujing; Yao, Bing; Shin, Jaehoon; Zhang, Feiran (2016-05-05). "Zika Virus Infects Human Cortical Neural Progenitors and Attenuates Their Growth". Cell Stem Cell. 18 (5): 587–590. doi:10.1016/j.stem.2016.02.016. ISSN 1934-5909.
![]() |
Wikimedia Commons has media related to Gyrification. |