Small interfering RNA
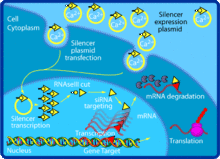
Small interfering RNA (siRNA), sometimes known as short interfering RNA or silencing RNA, is a class of double-stranded RNA molecules, 20-25 base pairs in length, similar to miRNA, and operates within the RNA interference (RNAi) pathway. It interferes with the expression of specific genes with complementary nucleotide sequences by degrading mRNA after transcription,[1] resulting in no translation.
siRNA can also act in RNAi-related pathways as an antiviral mechanism or play a role in the shaping of the chromatin structure of a genome. siRNAs and their role in post-transcriptional gene silencing (PTGS) were first discovered in plants by David Baulcombe's group at the Sainsbury Laboratory in Norwich, England and reported in Science in 1999.[2] Thomas Tuschl and colleagues soon reported in Nature that synthetic siRNAs could induce RNAi in mammalian cells.[3] This discovery led to a surge in interest in harnessing RNAi for biomedical research and drug development. Significant developments in siRNA therapies have been made with both organic (carbon based) and inorganic (non-carbon based) nanoparticles, such as these which have been successful in drug delivery to the brain, offering promising methods of delivery into human subjects. However, significant barriers to successful siRNA therapies remain, the most significant of which is off-targeting.[4]
Structure

siRNAs have a well-defined structure: a short (usually 20 to 24-bp) double-stranded RNA (dsRNA) with phosphorylated 5' ends and hydroxylated 3' ends with two overhanging nucleotides. The Dicer enzyme catalyzes production of siRNAs from long dsRNAs and small hairpin RNAs.[5] siRNAs can also be introduced into cells by transfection. Since in principle any gene can be knocked down by a synthetic siRNA with a complementary sequence, siRNAs are an important tool for validating gene function and drug targeting in the post-genomic era.
Mechanism
The mechanism by which siRNA causes gene silencing through repression of transcription occurs as follows:
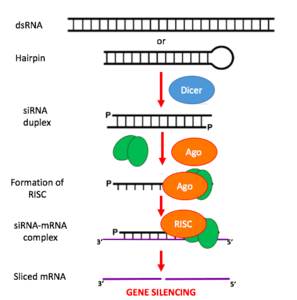
- Long dsRNA which can come from the following sources: hairpin, complementary RNAs, RNA dependent RNA polymerases. The long dsRNA is cleaved by an endo-ribonuclease called Dicer. Dicer cuts the long dsRNA to form short interfering RNA or siRNA.
- Once siRNA enters the cell it gets incorporated into other proteins to form the RNA-induced Silencing Complex or RISC.
- Once the siRNA is part of the RISC complex, the siRNA is unwound to form single stranded siRNA.
- One of the single stranded siRNAs is chosen at random to stay a part of the RISC complex.
- The single stranded siRNA which is part of the RISC complex now can scan and find a complementary mRNA
- Once the single stranded siRNA (part of the RISC complex) binds to its target mRNA, it induces mRNA cleavage.
- The mRNA is now cut and recognized as abnormal by the cell. This causes degradation of the mRNA and in turn no translation of the mRNA into amino acids and then proteins. Thus silencing the gene that encodes that mRNA.
siRNA is also similar to miRNA, however, miRNA silences genes by repression of translation, while siRNA works by cleaving the mRNA before translation.
RNAi induction using siRNAs or their biosynthetic precursors
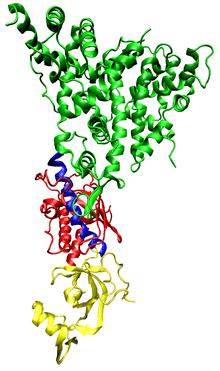
Gene knockdown by transfection of exogenous siRNA is often unsatisfactory because the effect is only transient, especially in rapidly dividing cells. This may be overcome by creating an expression vector for the siRNA. The siRNA sequence is modified to introduce a short loop between the two strands. The resulting transcript is a short hairpin RNA (shRNA), which can be processed into a functional siRNA by Dicer in its usual fashion.. Typical transcription cassettes use an RNA polymerase III promoter (e.g., U6 or H1) to direct the transcription of small nuclear RNAs (snRNAs) (U6 is involved in gene splicing; H1 is the RNase component of human RNase P). It is theorized that the resulting siRNA transcript is then processed by Dicer.
The gene knockdown efficiency can also be improved by using cell squeezing.[6]
The activity of siRNAs in RNAi is largely dependent on its binding ability to the RNA-induced silencing complex (RISC). Binding of the duplex siRNA to RISC is followed by unwinding and cleavage of the sense strand with endonucleases. The remaining anti-sense strand-RISC complex can then bind to target mRNAs for initiating transcriptional silencing.[7]
RNA activation
It has been found that dsRNA can also activate gene expression, a mechanism that has been termed "small RNA-induced gene activation" or RNAa. It has been shown that dsRNAs targeting gene promoters induce potent transcriptional activation of associated genes. RNAa was demonstrated in human cells using synthetic dsRNAs, termed "small activating RNAs" (saRNAs). It is currently not known whether RNAa is conserved in other organisms.[8]
siRNA post-transcriptional gene silencing
The siRNA induced post transcriptional gene silencing starts with the assembly of the RNA-induced silencing complex (RISC). The complex silences certain gene expression by cleaving the mRNA molecules coding the target genes. To begin the process, one of the two siRNA strands, the guide strand, will be loaded into the RISC while the other strand, the passenger strand, is degraded. Certain Dicer enzymes may be responsible of loading the guide strand into RISC.[9] Then, the siRNA scans for and direct RISC to perfectly complementary sequence on the mRNA molecules.[10] The cleavage of the mRNA molecules is thought to be catalyzed by the Piwi domain of Argonaute proteins of the RISC. The mRNA molecule is then cut precisely by cleaving the phosphodiester bond between the target nucleotides which are paired to siRNA residues 10 and 11, counting from the 5’end.[11] This cleavage results in mRNA fragments that are further degraded by cellular exonucleases. The 5’ fragment is degraded from its 3’ end by exosome while the 3’ fragment is degraded from its 5’ end by 5' -3' exoribonuclease 1(XRN1).[12] Dissociation of the target mRNA strand from RISC after the cleavage allow more mRNA to be silenced. This dissociation process is likely to be promoted by extrinsic factors driven by ATP hydrolysis.[11]
Sometimes cleavage of the target mRNA molecule do not happen. In some cases, the endonucleolytic cleavage of the phosphodiester backbone may be suppressed by mismatches of siRNA and target mRNA near the cleaving site. Other times, the Argonaute proteins of the RISC lack endonuclease activity even when the target mRNA and siRNA are perfectly paired.[11] In such cases, gene expression will be silenced by miRNA induced mechanism instead.[10]

siRNA role in transposon silencing
Small interfering RNAs are the main form of RNAi responsible for transposon silencing. Most notably through the ping-pong method, piwi-interacting RNAs (piRNA) – the largest class of small non-coding RNAs – repress active transposons in germline cells.[13] This method is analogous to activity of RNA-dependent RNA polymerases (RdRP) in worms and plants, enzymes not present in the genomes of flies and mammals.[14]
Challenges: avoiding nonspecific effects
Because RNAi intersects with a number of other pathways, it is not surprising that on occasion nonspecific effects are triggered by the experimental introduction of an siRNA. When a mammalian cell encounters a double-stranded RNA such as an siRNA, it may mistake it as a viral by-product and mount an immune response. Furthermore, because structurally related microRNAs modulate gene expression largely via incomplete complementarity base pair interactions with a target mRNA, the introduction of an siRNA may cause unintended off-targeting.
Innate immunity
Introduction of too much siRNA can result in nonspecific events due to activation of innate immune responses.[15] Most evidence to date suggests that this is probably due to activation of the dsRNA sensor PKR, although retinoic acid-inducible gene I (RIG-I) may also be involved. The induction of cytokines via toll-like receptor 7 (TLR7) has also been described. One promising method of reducing the nonspecific effects is to convert the siRNA into a microRNA. MicroRNAs occur naturally, and by harnessing this endogenous pathway it should be possible to achieve similar gene knockdown at comparatively low concentrations of resulting siRNAs. This should minimize nonspecific effects.
Off-targeting
Off-targeting is another challenge to the use of siRNAs as a gene knockdown tool. Here, genes with incomplete complementarity are inadvertently downregulated by the siRNA (in effect, the siRNA acts as a miRNA), leading to problems in data interpretation and potential toxicity. This, however, can be partly addressed by designing appropriate control experiments, and siRNA design algorithms are currently being developed to produce siRNAs free from off-targeting. Genome-wide expression analysis, e.g., by microarray technology, can then be used to verify this and further refine the algorithms. A 2006 paper from the laboratory of Dr. Khvorova implicates 6- or 7-basepair-long stretches from position 2 onward in the siRNA matching with 3'UTR regions in off-targeted genes.[16]
Adaptive immune responses
Plain RNAs may be poor immunogens, but antibodies can easily be created against RNA-protein complexes. Many autoimmune diseases see these types of antibodies. There haven’t yet been reports of antibodies against siRNA bound to proteins. Some methods for siRNA delivery adjoin polyethylene glycol (PEG) to the oligonucleotide reducing excretion and improving circulating half-life. However recently a large Phase III trial of PEGylated RNA aptamer against factor IX had to be discontinued by Regado Biosciences because of a severe anaphylactic reaction to the PEG part of the RNA. This reaction led to death in some cases and raises significant concerns about siRNA delivery when PEGylated oligonucleotides are involved.[17]
Chemical modification in siRNAs
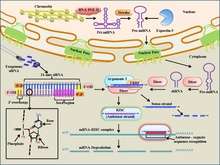
siRNAs have been chemically modified to enhance their therapeutic properties like enhanced activity, increased serum stability and less off-targets and decreased immunological activation. Detailed database of all such chemical modification is manually curated as siRNAmod in scientific literature.[18]
Therapeutic applications and challenges
Given the ability to knock down, in essence, any gene of interest, RNAi via siRNAs has generated a great deal of interest in both basic[19] and applied biology.
One of the biggest challenges to siRNA and RNAi based therapeutics is intracellular delivery.[20] Delivery of siRNA via nanoparticles has shown promise.[4][20] siRNA oligos in vivo are vulnerable to degradation by plasma and tissue nucleases [4] and have only shown mild effectiveness in localized delivery sites, like the human eye [21] Delivering pure DNA to target organisms is challenging because their large size and structure prevents them from diffusing readily across membranes.[20] siRNA oligos circumvent this problem due to their small size of 21-23 oligos.[22] This allows delivery via nano-scale delivery vehicles called nanovectors.[21]
A good nanovector for siRNA delivery should protect siRNA from degradation, enrich siRNA in the target organ and facilitate the cellular uptake of siRNA.[4] The three main groups of siRNA nanovectors are: lipid based, non-lipid organic-based, and inorganic.[4] Lipid based nanovectors are excellent for delivering siRNA to solid tumors,[4] but other cancers may require different non-lipid based organic nanovectors such as cyclodextrin based nanoparticles.[4][23]
siRNAs delivered via lipid based nanoparticles have been shown to have therapeutic potential for central nervous system (CNS) disorders [24] Central nervous disorders are prevalent but the blood brain barrier (BBB) often blocks access of potential therapeutics to the brain.[24] siRNAs that target and silence efflux proteins on the BBB surface have been shown to create an increase in BBB permeability.[24] siRNA delivered via lipid based nanoparticles are able to cross the BBB completely [24]
A huge problem in siRNA delivery is the issue of off-targeting.[20][21] Since genes are read in both directions, there exists a possibility that even if the intended antisense siRNA strand is read and knocks out the target mRNA, the sense siRNA strand may target another protein involved in another function.[25]
Phase I results of the first two therapeutic RNAi trials (indicated for age-related macular degeneration, aka AMD) reported at the end of 2005 that siRNAs are well tolerated and have suitable pharmacokinetic properties.[26]
In a phase 1 clinical trial, 41 patients with advanced cancer metastasised to liver were administered with RNAi delivered through lipid nanoparticles. The RNAi targeted two genes encoding key proteins in the growth of the cancer cells, vascular endothelial growth factor, (VEGF), and kinesin spindle protein (KSP). The results showed clinical benefits, with the cancer either stabilized after six months or regression of metastasis in some of the patients. Pharmacodynamics analysis of biopsy samples from the patients revealed the presence of the RNAi constructs in the samples, proving that the molecules reached the intended target.[27][28]
Proof of concept trials have indicated that Ebola-targeted siRNAs may be effective as post-exposure prophylaxis in humans, with 100% of non-human primates surviving a lethal dose of Zaire Ebolavirus, the most lethal strain.[29]
Intracellular Delivery
Delivering siRNA intracellular continues to be a challenge. There are three main techniques of delivery for siRNA that differ on efficiency and toxicity.
Transfection
In this technique siRNA first must be designed against the target gene. Once the siRNA is configured against the gene it has to be effectively delivered through a transfection protocol. Delivery is usually done by cationic liposomes, polymer nanoparticles, and lipid conjugation. This method is advantageous because it can deliver siRNA to most types of cells, has high efficiency and reproducibility, and is offered commercially. The most common commercial reagents for transfection of siRNA are Lipofectamine and Neon Transfection. However it is not compatible with all cell types, and has low in vivo efficiency.[30][31]
Electroporation
Electrical pulses are also used to intracellularly deliver siRNA into cells. The cell membrane is made of phospholipids which makes it susceptible to an electric field. When quick but powerful electrical pulses are initiated the lipid molecules reorient themselves, while undergoing thermal phase transitions because of heating. This results in the making of hydrophilic pores and localized perturbations in the lipid bilayer cell membrane also causing a temporary loss of semipermeability. This allows for the escape of many intracellular contents, such as ions and metabolites as well as the simultaneous uptake of drugs, molecular probes, and nucleic acids. For cells that are difficult to transfect electroporation is advantageous however cell death is more probable under this technique.[32]
This method has been used to deliver siRNA targeting VEGF into the xenografted tumors in nude mice, which resulted in a significant suppression of tumor growth.[33]
Viral-mediated delivery
The gene silencing effects of transfected designed siRNA are generally transient, but this difficulty can be overcome through an RNAi approach. Delivering this siRNA from DNA templates can be done through several recombinant viral vectors based on retrovirus, adeno-associated virus, adenovirus, and lentivirus.[34] The latter is the most efficient virus that stably delivers siRNA to target cells as it can transduce nondividing cells as well as directly target the nucleus.[35] These specific viral vectors have been synthesized to effectively facilitate siRNA that is not viable for transfection into cells. Another aspect is that in some cases synthetic viral vectors can integrate shRNA into the cell genome which allows for stable expression of siRNA and long-term gene knockdown. This technique is advantageous because it is in vivo and effective for difficult to transfect cell. However problems arise because it can trigger antiviral responses in some cell types leading to mutagenic and immunogenic effects.
This method has potential use in gene silencing of the central nervous system for the treatment of Huntington’s disease.[36]
See also
- Gene knockdown
- Gene silencing
- Oligonucleotide synthesis
- EsiRNA
- NatsiRNA
- MicroRNA
- Viroid
- RNA interference
- CRISPR
- Dharmacon
- Persomics
References
- ↑ RNA Interference: Biology, Mechanism, and Applications
- ↑ Hamilton A, Baulcombe D (1999). "A species of small antisense RNA in posttranscriptional gene silencing in plants". Science. 286 (5441): 950–2. doi:10.1126/science.286.5441.950. PMID 10542148.
- ↑ Elbashir S, Harborth J, Lendeckel W, Yalcin A, Weber K, Tuschl T (2001). "Duplexes of 21-nucleotide RNAs mediate RNA interference in cultured mammalian cells". Nature. 411 (6836): 494–988. doi:10.1038/35078107. PMID 11373684.
- 1 2 3 4 5 6 7 Nanovector delivery of siRNA for cancer therapy. H Shen, T Sun and M Ferrari. Review. Cancer Gene Therapy (2012) 19, 367-373; doi: 10.1038/cgt.2012.22;published online 4 May 2012
- ↑ Bernstein E, Caudy A, Hammond S, Hannon G (2001). "Role for a bidentate ribonuclease in the initiation step of RNA interference". Nature. 409 (6818): 363–6. doi:10.1038/35053110. PMID 11201747.
- ↑ Armon Sharei; Janet Zoldan; Andrea Adamo; Woo Young Sim; Nahyun Cho; Emily Jackson; Shirley Mao; Sabine Schneider; Min-Joon Han; Abigail Lytton-Jean; Pamela A. Basto; Siddharth Jhunjhunwala; Jungmin Lee; Daniel A. Heller; Jeon Woong Kang; George C. Hartoularos; Kwang-Soo Kim; Daniel G. Anderson; Robert Langer & Klavs F. Jensen (2013). "A vector-free microfluidic platform for intracellular delivery". PNAS. 110 (6): 2082–7. doi:10.1073/pnas.1218705110. PMC 3568376
. PMID 23341631.
- ↑ Daneholt, B. (2006). "Advanced Information: RNA interference". The Novel Prize in Physiology or Medicine.
- ↑ Li LC (2008). "Small RNA-Mediated Gene Activation". RNA and the Regulation of Gene Expression: A Hidden Layer of Complexity. Caister Academic Press. ISBN 978-1-904455-25-7.
- ↑ Lee, Young Sik; Nakahara, Kenji; Pham, John W; Kim, Kevin; He, Zhengying; Sontheimer, Erik J; Carthew, Richard W. "Distinct Roles for Drosophila Dicer-1 and Dicer-2 in the siRNA/miRNA Silencing Pathways". Cell. 117 (1): 69–81. doi:10.1016/s0092-8674(04)00261-2.
- 1 2 Carthew, Richard W.; Sontheimer, Erik J. "Origins and Mechanisms of miRNAs and siRNAs". Cell. 136 (4): 642–655. doi:10.1016/j.cell.2009.01.035. PMC 2675692
. PMID 19239886.
- 1 2 3 Tomari, Yukihide; Zamore, Phillip D. (2005-03-01). "Perspective: machines for RNAi". Genes & Development. 19 (5): 517–529. doi:10.1101/gad.1284105. ISSN 0890-9369. PMID 15741316.
- ↑ Orban, Tamas I.; Izaurralde, Elisa (2005-04-01). "Decay of mRNAs targeted by RISC requires XRN1, the Ski complex, and the exosome". RNA. 11 (4): 459–469. doi:10.1261/rna.7231505. ISSN 1355-8382. PMC 1370735
. PMID 15703439.
- ↑ Moazed, Danesh. "Small RNAs in transcriptional gene silencing and genome defence". Nature. 457 (7228): 413–420. doi:10.1038/nature07756. PMC 3246369
. PMID 19158787.
- ↑ Siomi, Mikiko C.; Sato, Kaoru; Pezic, Dubravka; Aravin, Alexei A. "PIWI-interacting small RNAs: the vanguard of genome defence". Nature Reviews Molecular Cell Biology. 12 (4): 246–258. doi:10.1038/nrm3089.
- ↑ Whitehead, K. A.; Dahlman, J. E.; Langer, R. S.; Anderson, D. G. (2011). "Silencing or Stimulation? SiRNA Delivery and the Immune System". Annual Review of Chemical and Biomolecular Engineering. 2: 77–96. doi:10.1146/annurev-chembioeng-061010-114133. PMID 22432611.
- ↑ Birmingham A, Anderson E, Reynolds A, Ilsley-Tyree D, Leake D, Fedorov Y, Baskerville S, Maksimova E, Robinson K, Karpilow J, Marshall W, Khvorova A (2006). "3' UTR seed matches, but not overall identity, are associated with RNAi off-targets". Nat Methods. 3 (3): 199–204. doi:10.1038/nmeth854. PMID 16489337.
- ↑ Wittrup, Anders; Lieberman, Judy. "Knocking down disease: a progress report on siRNA therapeutics". Nature Reviews Genetics. 16 (9): 543–552. doi:10.1038/nrg3978. PMC 4756474
. PMID 26281785.
- ↑ Dar, Showkat; et al. (2016). "siRNAmod: A database of experimentally validated chemically modified siRNAs". Scientific Reports. 6: 20031. doi:10.1038/srep20031. PMC 4730238
. PMID 26818131. Retrieved 2016. Check date values in:
|access-date=
(help) - ↑ Alekseev OM, Richardson RT, Alekseev O, O'Rand MG (2009). "Analysis of gene expression profiles in HeLa cells in response to overexpression or siRNA-mediated depletion of NASP". Reproductive Biology and Endocrinology. 7: 45. doi:10.1186/1477-7827-7-45. PMC 2686705
. PMID 19439102.
- 1 2 3 4 Promise and Challenge of RNA Interference-Based Therapy for Cancer. Petrocca, Fabio and Lieberman, Judy. Journal of Oncology. Biology of Neoplasia. Volume 29, number 6, February 20, 2011.
- 1 2 3 RNA-Based Therapeutics: Current Progress and Future Prospects. John C. Burnett and John J. Rossi. Cell Press Chemistry and Biology Review. Department of Molecular and Cellular Biology, Beckman Research Institute of the City of Hope, Duarte, CA 91010, USA. doi:10.1016/j.chembiol.2011.12.008
- ↑ Elbashir SM, Lendeckel W, Tuschl T. RNA interference is mediated by 21- and 22-nucleotide RNAs. Genes Dev. Jan 15; 2001 15(2):188–200. [PubMed: 11157775]
- ↑ Heidel JD, Yu Z, Liu JY, Rele SM, Liang Y, Zeidan RK, et al. Administration in non-human primates of escalating intravenous doses of targeted nanoparticles containing ribonucleotide reductase subunit M2 siRNA. Proc Natl Acad Sci U S A. Apr 3; 2007 104(14):5715–21. [PubMed: 17379663]
- 1 2 3 4 Gomes, Dreier, Brewer et. al. A new approach for a blood-brain barrier model based on phospholipid vesicles: Membrane development and siRNA-loaded nanoparticles permability. Journal of Membrane Science. Volume 503. p.8-15. Published: March 2016.
- ↑ Peptides Used in the Delivery of Small Noncoding RNA. Ravi S. Shukla, Bin Qin, Kun Cheng. Molecular Pharmaceutics. 2014 Oct 6; 11(10): 3395-3408. Published online 2014 Aug 26. Doi: 10..1021/mp500426r.
- ↑ Tansey B (11 August 2006). "Macular degeneration treatment interferes with RNA messages". San Francisco Chronicle.
- ↑ Vall D’Hebron Institute of Oncology (11 February 2013). "First-in-man study demonstrates the therapeutic effect of RNAi gene silencing in cancer treatment". Retrieved 31 May 2013.
- ↑ Tabernero, Josep; Shapiro, Geoffrey; LoRusso, Patricia; Cervantes, Andres; Schwartz, Gary; Weiss, Glen; Paz-Ares, Luis; Cho, Daniel; et al. (2013). "First-in-Humans Trial of an RNA Interference Therapeutic Targeting VEGF and KSP in Cancer Patients with Liver Involvement". Cancer Discovery. 3 (4): 406–417. doi:10.1158/2159-8290.CD-12-0429. PMID 23358650.
- ↑ Postexposure protection of non-human primates against a lethal Ebola virus challenge with RNA interference: a proof-of-concept study Prof Thomas W Geisbert PhD,Amy CH Lee MSc,Marjorie Robbins PhD,Joan B Geisbert,Anna N Honko PhD,Vandana Sood MSc,Joshua C Johnson BSc,Susan de Jong PhD,Iran Tavakoli BSc,Adam Judge PhD,Lisa E Hensley PhD,Ian MacLachlan PhD The Lancet - 29 May 2010 ( Vol. 375, Issue 9729, Pages 1896-1905 ) doi:10.1016/S0140-6736(10)60357-1 PMID 20511019
- ↑ Jensen, Kirsty; Anderson, Jennifer A.; Glass, Elizabeth J. (2014-04-15). "Comparison of small interfering RNA (siRNA) delivery into bovine monocyte-derived macrophages by transfection and electroporation". Veterinary Immunology and Immunopathology. 158 (3–4): 224–232. doi:10.1016/j.vetimm.2014.02.002. PMC 3988888
. PMID 24598124.
- ↑ Chatterjea, MN (2012). Textbook of Medical Biochemistry. New Delhi: Jaypee Brothers Medical Publishers. p. 304 – via 8 ed.
- ↑ "siRNA Delivery Methods into Mammalian Cells". 2016-10-13.
- ↑ Takei, Yoshifumi (2014-01-01). Li, Shulin; Cutrera, Jeffry; Heller, Richard; Teissie, Justin, eds. Electroporation Protocols. Methods in Molecular Biology. Springer New York. pp. 131–138. doi:10.1007/978-1-4614-9632-8_11. ISBN 9781461496311.
- ↑ Talwar, G.P; Hasnain, Seyed; Sarin, Shiv Kumar (January, 2016). TEXTBOOK OF BIOCHEMISTRY, BIOTECHNOLOGY, ALLIED AND MOLECULAR MEDICINE (4 ed.). PHI Learning Private Limited. p. 873. ISBN 978-81-203-5125-7.
- ↑ Morris, K. V.; Rossi, J. J. (2006-01-05). "Lentiviral-mediated delivery of siRNAs for antiviral therapy". Gene Therapy. 13 (6): 553–558. doi:10.1038/sj.gt.3302688. ISSN 0969-7128.
- ↑ Cambon, Karine; Déglon, Nicole (2013-01-01). Kohwi, Yoshinori; McMurray, Cynthia T., eds. Trinucleotide Repeat Protocols. Methods in Molecular Biology. Humana Press. pp. 95–109. doi:10.1007/978-1-62703-411-1_7. ISBN 9781627034104.
Further reading
- Hannon, G. J.; Rossi, J. J. (2004). "Unlocking the potential of the human genome with RNA interference". Nature. 431 (7006): 371–378. Bibcode:2004Natur.431..371H. doi:10.1038/nature02870. PMID 15372045.
External links
![]() |
Wikimedia Commons has media related to Small interfering RNA. |
- An animation of the mechanism of siRNA by Nature Reviews Genetics
- DesiRM: Designing of Complementary and Mismatch siRNAs for Silencing a Gene